Study Demonstrates Quantum Entanglement Between Light And Microwave Photons
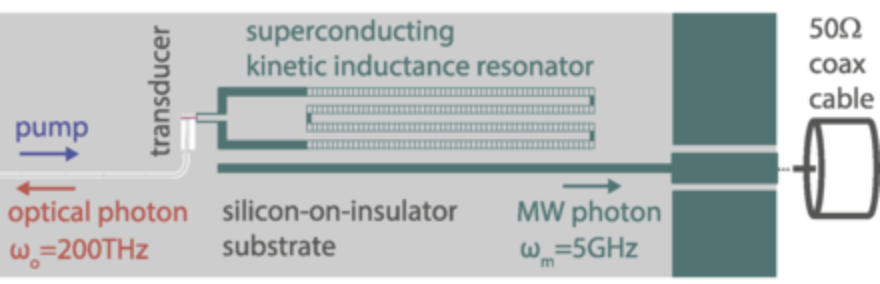
Insider Brief
- Researchers demonstrated entanglement between light and microwave photons, a key step to connect quantum networks with distant quantum processors.
- The team developed a chip-scale device using acoustic transducers to generate entangled photon pairs at cryogenic temperatures.
- Despite challenges like pump-induced noise, the study lays the groundwork for future quantum communication systems and modular quantum computing architectures.
Researchers report they have developed a novel source of entangled photons in a step toward more robust quantum communication and computation architectures.
Specifically, the study, published in Physical Review X, demonstrates entanglement between light and microwave photons separated by five orders of magnitude in energy, paving the way for quantum networks connecting superconducting processors.
“Our work demonstrates a fundamental prerequisite for a quantum information processing architecture in which room-temperature optical communication links may be used to network superconducting quantum-bit processors in distant cryogenic setups,” the team writes.
The team, composed of researchers from institutions such as the California Institute of Technology and the University of Chicago, focused on overcoming challenges in creating efficient quantum transducers. These devices are critical for converting quantum states between different mediums, such as optical and microwave photons, for long-distance quantum communication.
However, earlier efforts faced obstacles like low efficiency and noise, which hindered practical implementation. By designing a chip-scale device with an integrated acoustic transducer, the researchers achieved entanglement between optical and microwave photons, which is crucial for future quantum networks. Acoustic transducers — simple types are found in microphones and speakers — convert sound waves, which is acoustic energy, into electrical signals, or vice versa, and can modulate frequencies.
This entanglement allows for reliable quantum communication over long distances using optical channels while preserving the computational advantages of superconducting processors.
Extending The Capability
Entanglement, a core feature of quantum computing, enables secure communication and complex quantum information tasks. Historically, entangled light sources were developed to explore quantum mechanics fundamentals. In this study, the researchers extended this capability by engineering entangled photons across vastly different energy levels, an innovation that holds promise for quantum networks and distributed sensing applications.
As quantum processors grow in size and complexity, future systems will likely rely on modular architectures that connect different quantum devices through communication links, according to the researchers
The entanglement source provides a building block for creating modular quantum systems, connecting superconducting quantum processors via optical channels, according to the scientists.
This would be in line with the future direction of superconducting quantum computing, the they add: “The growing size and complexity of superconducting quantum processors suggests that future, large-scale quantum computers based on superconducting qubits will likely be modular systems with interconnected processors. Toward this long-term goal, a quantum network architecture has been envisioned with optical channels as low-loss, room-temperature communication links between superconducting processors cooled in separate dilution refrigerator nodes.”
The network they envision would use low-loss, room-temperature optical links to connect processors housed in cryogenic environments. This advance could help scale quantum computing systems, especially in areas such as secure communication and large-scale computation.
Methods and Key Findings
The core of the researchers’ approach involved an acoustic transducer, in this case, a device that simultaneously modulates optical cavity frequencies and generates voltage in superconducting electrical resonators. The experiment was conducted at cryogenic temperatures to maintain near-quantum ground states for the system’s components. Using laser pulses, the team generated entangled photon pairs and measured the statistical correlations between microwave and optical emissions to confirm entanglement.
One of the significant findings is that the device’s performance exceeds classical limits, indicating successful entanglement. The Bell state fidelity exceeded the classical limit by over four standard deviations, demonstrating entanglement between microwave-optical states, according to the researchers.
Despite some limitations due to pump-induced noise, the results suggest that this method could form the basis for high-fidelity quantum operations in a distributed network.
The microwave photonic qubits generated in the experiment can directly interact with superconducting qubits, facilitating quantum teleportation and other advanced protocols. Additionally, the researchers noted that the approach is resilient to certain types of quantum errors, such as loss errors, making it a robust option for future quantum systems.
Limitations and Future Directions
While the experiment demonstrates a major step forward, there are still several challenges to address. The primary source of noise in the system comes from pump-induced heating, which reduces the fidelity of the entangled states. The researchers aim to improve the thermal management of the transducer to increase entanglement rates and fidelity in future experiments. They add that by improving the thermalization of the transducer, we can further enhance the system’s performance and increase entanglement generation rates to the order of 1,000 per second.
Future research will also focus on integrating the system with superconducting qubit nodes to create functional quantum networks.
The study represents a collaborative effort across multiple fields of quantum research, combining expertise in optomechanics, superconducting circuits and quantum information science. The team includes Srujan Meesala, David Lake and Steven Wood, with contributions from Piero Chiappina, Changchun Zhong, Andrew D. Beyer, Matthew D. Shaw, Liang Jiang and Oskar Painter. The researchers are affiliated with leading institutions such as the California Institute of Technology, the University of Chicago and the Jet Propulsion Laboratory.