Harnessing the Power of Neutrality: Comparing Neutral-Atom Quantum Computing With Other Modalities
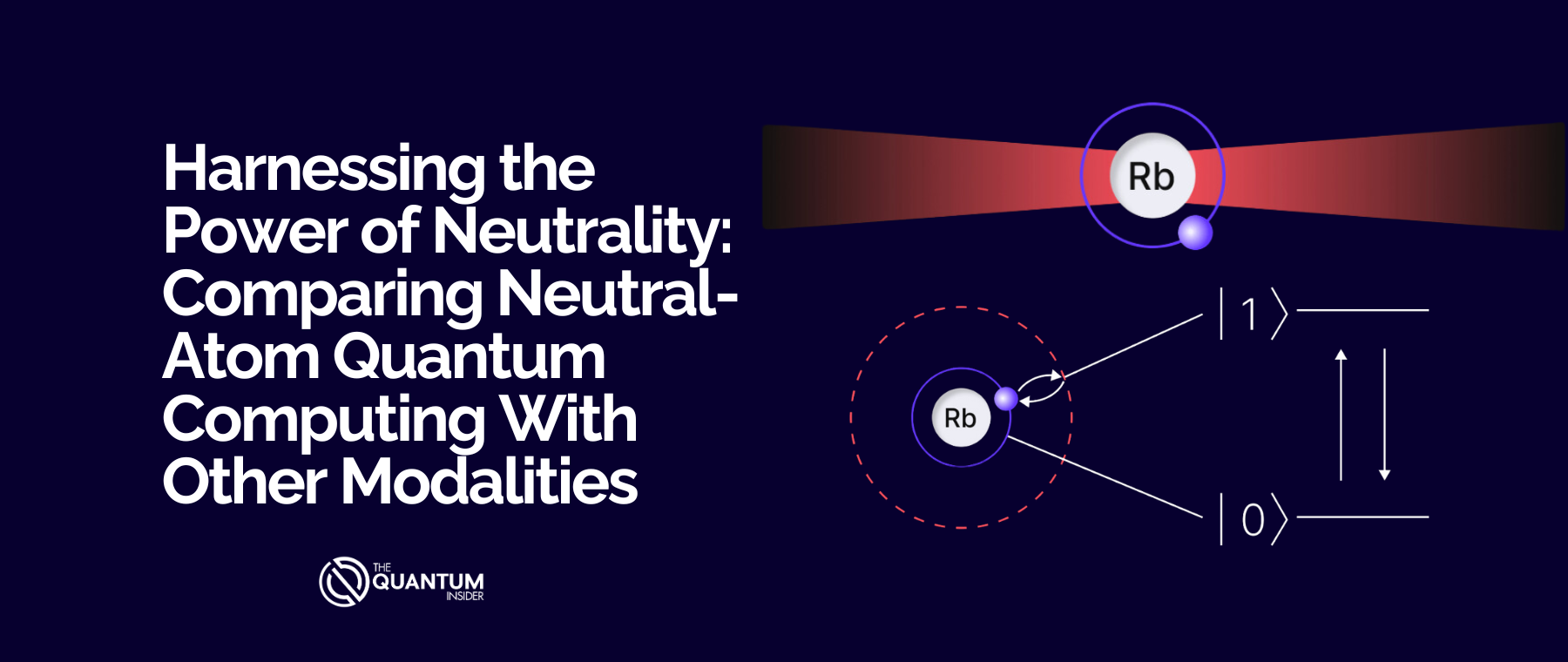
Quantum computing promises to dramatically increase processing power to tackle certain types of computational challenges, leveraging the strange and counterintuitive properties of quantum mechanics. What many people do not understand is that there is not just one type of quantum computer. In fact, scientists and entrepreneurs have developed several variations that are based on different approaches, or modalities.
News that a Harvard-led team of scientists relied on a neutral-atom quantum computer to execute large-scale algorithms using 48 logical qubits shifted that modality into the spotlight. In fact, the experiment is just the latest advance for neutral atom computing, which is emerging as a leading way to tap the power of quantum computation to solve the world’s most complex problems and unravel its deepest scientific mysteries.
The neutral atom approach to quantum computing uses individual, laser-cooled neutral atoms as quantum bits, or qubits, which are the quantum version of the classical bit. However, the principles of quantum mechanics offer qubits much vaster computational possibilities.
In the neutral atom approach, the qubits are manipulated and held in place by optical tweezers. This approach allows for several advantages, including precise control and scalable quantum information processing, that go to the heart of developing quantum computers that can be used in real-world applications, according to Yuval Boger, Chief Marketing Officer for QuEra Computing, a leader in neutral-atom quantum computing and a partner with Harvard in the study that led to the advance in logical qubits.
“Neutral-atom quantum computing offers several advantages over other modalities. Atoms are ‘nature’s perfect qubits’, perfectly identical without manufacturing defects,” said Boger. “These systems can operate at room temperature, simplifying installation and reducing power consumption. The ability to shuttle qubits while maintaining their quantum state facilitates efficient algorithms and error correction. Additionally, neutral-atom platforms scale with fewer control signals and do not require optical interconnects at least until reaching 10,000 qubits. Collectively, these attributes make neutral atoms a very attractive quantum computing modality.”
That sentiment is echoed by Dr. Mukund Vengalattore, program manager of the Defense Sciences Office (DSO) at the Defense Advanced Research Project Agency (DARPA). Vengalattore said the purity of the rubidium atom, which lies at the heart of the neutral atom approach, is one reason for confidence in the modality.
“Every atom of rubidium is identical to every other item of rubidium in the universe,” said Vengalattore. “There are no fabrication imperfections, there are no errors in how you compose a rubidium atom – nature does that for us at a level that we can’t even imagine. So when we trap these atoms, and we control them, and we measure their properties, we know that every rubidium atom in a certain sense acts exactly as every other rubidium atom.”
DARPA provided funding for the Harvard-QuEra study through its Optimization with Noisy Intermediate-Scale Quantum Devices (ONISQ) program.
How Do Quantum Computing Compute?
To understand the various modalities of quantum computing and how the neutral atom approach compares to these other variations, it’s helpful to first grasp how quantum computers differ from classical computers and how other approaches differ from the neutral atom approach.
Classical computers, like the laptop or phone you’re using right now, process information in bits that are either 0s or 1s. Think of the computational potential as a super-fast game of twenty questions, with traditional algorithms designed to find speedy answers to yes and no questions.
Quantum computers, as we mentioned, use quantum bits or qubits, which can be in a state of 0, 1, or a “superposition” of these states. With entanglement – the connection between particles where the state of one particle influences the state of the other – operations performed on one entangled qubit are correlated with other qubits, giving quantum computers the potential to perform high-speed, complex calculations.
Essentially, by tapping into superposition and entanglement, quantum computers can use the probabilities to play that game of twenty questions even faster – potentially much, much faster – than our laptops, phones and even existing supercomputers.
But notice, when we refer to the immense power of quantum computers, they tend to use words like “potential” or “possible.” That’s because there are challenges associated with using quantum computers for everyday tasks, like balancing portfolios, searching for the best pharmaceuticals to treat diseases, or, maybe even playing twenty million questions, the quantum version of that game.
The first challenge, as you might have guessed, is that quantum superposition and entanglement are extremely sensitive states. Environmental noise – from heat to magnetic waves to cosmic rays – can interfere with these sensitive quantum processes, creating errors in calculations that must be corrected and mitigated, which, quite accurately, are described as quantum error correction and quantum error mitigation.
With all these limitations in mind, scientists have quantum computers built using different physical systems and operate on various principles to manage error correction and error mitigation. However, although some of these approaches limit errors, they may cause other problems. For example, engineers may deal with errors by designing complex cooling systems and intricate wiring, but that can also limit the practicality of those quantum computers.
Quantum Modalities – Pros and Cons
Here’s an overview of the key modalities of quantum computing and a list of their currently assessed strengths and vulnerabilities. It’s important to recognize that comparisons across the modalities often lack technological nuance and do not consider the varieties of technological maturity and varying levels of progress that could rapidly alter these performance standards.
Superconducting Quantum Computers
Superconducting qubits harness the phenomenon of superconductivity to perform quantum computations. Superconductivity, a state achieved at extremely low temperatures, allows electrical current to flow without resistance. This property is pivotal for creating qubits with minimal energy loss, a crucial factor for maintaining quantum coherence over operational timescales. Superconducting qubits are fabricated using materials like aluminum or niobium, which become superconducting when cooled near absolute zero. These qubits operate by creating a quantized magnetic flux or charge states that represent the 0 and 1 of quantum bits, exploiting the Josephson junction—a non-linear superconducting electrical junction that enables control and readout of the qubit state through microwave pulses. The precise manipulation of these pulses allows for the execution of quantum logic operations, essential for quantum computing.
Strengths include:
- Speed: These systems operate at extremely fast timescales (nanoseconds), and are thus capable of performing millions of gate operations per second, leading to swift computations.
- Commercial: Leading the race in terms of commercially available quantum processors.
- Control: They have advanced in terms of the precision with which qubits can be controlled.
Weaknesses include:
- Sensitive: Superconducting qubits are extremely sensitive to external noise and lose their quantum state quickly (decoherence).
- Cooling Requirements: They typically require very low temperatures to operate, close to absolute zero, which involves complex and expensive equipment.
- Wiring: These devices also require intricate wiring, and the amount of wiring increases linearly with the number of qubits.
- Expense: Superconducting quantum computers can be expensive both to build and maintain.
- Connectivity: superconducting qubits are fixed in place. A given qubit can only interact with a few neighboring qubits. This increases the number of operations that need to be performed to complete an algorithm and limits the choice of error correction algorithms.
- Scalability concerns: beyond several hundred qubits, many vendors assume that optical interconnects will be required to connect several computing units. The transition to and from the optical interconnect adds cost and complexity.
Trapped Ion Quantum Computers
Trapped ion quantum computers operate by confining charged atoms, or ions, in an electromagnetic field, leveraging the ions’ quantum states to function as qubits. This method utilizes the inherent quantum mechanical properties of ions, such as energy levels, to encode information. Each ion’s electron can be excited to different energy levels, representing the qubit states. Quantum logic operations are performed by precisely targeting these ions with lasers, which manipulate their quantum states through excitation and induce entanglement between qubits via their Coulomb interaction.
Strengths include:
- High Fidelity: Trapped ions have longer coherence times, allowing for longer calculations before qubits lose their quantum state.
- Fully Connected Network: They naturally allow for ions to interact with each other, enabling a fully connected network of qubits.
- Long coherence times: Information can persist for minutes, enabling extended computations.
Weaknesses include:
- Speed: Operations with trapped ions are typically slower than with superconducting qubits.
- Engineering Complexity: Building and scaling up trapped ion systems are technically challenging.
- Scalability challenges: Trapping a large number of ions efficiently remains a hurdle.
- Complex control systems: Precise manipulation of individual ions requires sophisticated laser setups.
- Bulkiness: Current setups are large and require specialized infrastructure.
Silicon Quantum Computers
Silicon qubits leverage the well-established techniques of the semiconductor industry. These qubits are fabricated using silicon-based materials, similar to those used in traditional computer chips, but operate on quantum mechanical principles. The key to silicon qubits lies in their use of either the spin of a single electron trapped in a silicon-based quantum dot or the nuclear spin of phosphorus atoms embedded in a silicon matrix. By applying precise electrical or magnetic fields, the spin states of these particles can be controlled, allowing them to represent the 0 and 1 states of qubits.
Strengths include:
- Compatibility with Existing Technology: Silicon-based qubits could be manufactured with techniques similar to those used for classical computer chips.
- Potential for miniaturization: Compact devices are achievable due to the well-understood nature of silicon technology.
- Room-temperature operation: Potentially eliminates the need for complex cryogenic systems.
Weaknesses include:
- Control Difficulty: Individual qubits can be hard to control and read out, which presents a significant challenge.
- Very short coherence times: Information loss occurs within nanoseconds, significantly limiting computation length.
- Sensitivity to noise: Highly susceptible to environmental noise, requiring sophisticated shielding techniques.
Photonic Quantum Computers
Photonic qubits utilize light particles, or photons, to carry quantum information. Unlike methods that rely on matter-based qubits, photonic quantum computing harnesses the quantum properties of light, such as polarization or phase, to encode information in qubits. This technique involves generating, manipulating, and measuring photons through optical components like beam splitters, mirrors, and phase shifters, integrated into optical circuits. These components allow for the precise control of the path and properties of photons, enabling the execution of quantum logic operations without the need for the ultra-cold temperatures required by other quantum computing platforms.
Strengths include:
- Warm Temperature Operations: Photonic quantum computers are designed to operate in warmer temperatures and possibly room temperature, unlike many other qubit systems.
- Integration with Communication Technology: Photons are already used in fiber-optic cables, making them suitable for quantum communication.
- Naturally long coherence times: Photons inherently experience minimal decoherence, leading to extended information lifetimes.
Weaknesses include:
- Error Correction: Building an effective error correction system for photons is challenging.
- Loss and Noise: Photonic systems can suffer from loss and noise that impact the fidelity of the operations.
- Challenging gate operations: Implementing logic gates on light particles is complex and resource-intensive.
- Limited controllability: Addressing and manipulating individual photons presents significant difficulties.
In conclusion, each quantum computing modality has its own set of advantages and challenges. The ongoing research and development in this field aim to harness these unique strengths while mitigating the weaknesses. The ultimate goal is to build practical and reliable quantum computers that can tackle problems beyond the reach of classical machines, which would herald a new era in computation and technology.
How Does The Neutral Atom Approach Compare
The neutral atom approach is a well-known and extensively investigated approach to quantum computing. The approach offers numerous advantages, especially in terms of scalability, expense, error mitigation, error correction, coherence, and simplicity.
Neutral atom quantum computing utilizes individual atoms, typically alkali atoms like rubidium or cesium, suspended and isolated in a vacuum and manipulated using precisely targeted laser beams. These atoms are not ionized, meaning they retain all their electrons and do not carry an electric charge, which distinguishes them from trapped ion approaches. The quantum states of these neutral atoms, such as their energy levels or the orientation of their spins, serve as the basis for qubits. By employing optical tweezers—focused laser beams that trap and hold the atoms in place—arrays of atoms can be arranged in customizable patterns, allowing for the encoding and manipulation of quantum information.
Unlike charged particles or superconducting circuits, neutral atoms are less perturbed by external electromagnetic fields, reducing error rates, a significant challenge in quantum computing. The physical separation of atoms in the neutral atom approach also simplifies the implementation of quantum error correction protocols, which are crucial for creating fault-tolerant quantum computers.
The atoms are cooled and held in place using laser beams, a process that requires far less cooling than the deep cryogenic temperatures needed for superconducting circuits. While approaches, such as superconducting qubits operate near absolute zero, neutral atom quantum computers use laser cooling techniques to reach only a few microkelvins above absolute zero. This distinction means that the elaborate and energy-intensive infrastructure necessary to achieve and maintain millikelvin temperatures in superconducting systems is not needed for neutral atom quantum computing.
The scalability of neutral atom quantum computing is another major benefit. Atoms are arranged in two-dimensional arrays and manipulated with precision, allowing for the practical construction of large qubit arrays. Optical tweezers, which are focused beams of light, can trap and move individual atoms with high precision, facilitating the complex interactions required for quantum computations.
Neutral atoms also enjoy all-to-all connectivity. The ability to shuttle qubits, or move them without damaging their quantum state, means that every qubit can be shuttled close enough to interact with every other qubit. Such shuttling allows creating more efficient algorithms, and also enables new error correction techniques that are not available for modalities with static qubits.
Furthermore, neutral atom systems exhibit longer coherence times compared to other quantum systems. This means that they can perform complex quantum operations for longer periods before quantum information is degraded, enhancing computational capabilities.
In terms of technological requirements, neutral atom quantum computing systems are less demanding. They do not need the same degree of extreme miniaturization as nanofabricated circuits, nor the strict vacuum and temperature conditions of ion traps, potentially reducing the cost and complexity of quantum computing systems.
Historically, neutral atoms did not exhibit gate fidelities that were as high as superconducting or trapped ions. Higher gate fidelities translate to lower error rates and the ability to create longer and more complex circuits. However, recent developments have brought the fidelities of neutral atom approaches to be on par with their counterparts.
One disadvantage of neutral atom approaches is that the gate operations are slower than in superconducting qubits. This is because physical qubit movement (qubit shuttling) is involved both in the initial setup of the system as well as in moving qubits near each other so that they can be entangled. Several approaches have been suggested to overcome this limitation.
In conclusion, the exploration of various quantum computing modalities, from superconducting qubits to trapped ions, silicon qubits, and photonic systems, underscores the rich diversity of approaches in the quest for quantum supremacy. Among these, the neutral atom approach emerges as a particularly promising contender, offering unique advantages in scalability, error mitigation, and operational simplicity. As highlighted by the recent advancements and expert insights, each modality carries its own set of strengths and challenges. The journey towards practical and scalable quantum computing is complex and multifaceted, yet the ongoing innovations and collaborative efforts across modalities signal a bright horizon for quantum technologies.